Microbial Inoculants
By Barbara Bellows, Texas Institute for Applied Environmental Research; Mike Morris, NCAT; and Colin Mitchell, NCAT
Abstract
A growing number of companies are selling biofertilizers or biostimulants: microbial inoculants intended to enhance soil health. In laboratory and greenhouse studies, the microbes contained in these products have proven effective in enhancing nutrient availability, decreasing pest infestations, stimulating plant growth, protecting plants against stress conditions, and enhancing plant competition against invasive species. These benefits have not always been seen by farmers in “real world” conditions, however—making the value and cost-effectiveness of these products a topic of considerable uncertainty.
To help you reach your own conclusions, this publication explains how microbial inoculants work, reviews current (in 2020) scientific literature and studies, and offers practical recommendations: factors to consider before buying and using these products.
Contents
Introduction
Overview of Plant-Beneficial Microorganisms
Reasons Why Microbial Inoculants May Not Work
Overcoming Microbial Inoculant Limitations
Summary, Future Directions, and Recommendations
References
Further Resources
Introduction
A vast number of soil microorganisms influence nutrient uptake, water access, disease resistance, and many other factors related to plant growth and health. For example, some bacteria, fungi, and viruses have been shown to increase competition against weeds, while others can remediate or immobilize soil contaminants, including heavy metals (Mishra and Sarma, 2017) and hydrocarbons (Janczak et al., 2018).
Intense land use, tillage, and synthetic agricultural inputs can greatly diminish the number, diversity, and benefits provided by soil microorganisms. Many farmers and ranchers who want to restore plant-microbial interactions are exploring microbial inoculants: powders or solutions containing live or dormant microorganisms. Unlike chemical fertilizers that contain plant nutrients (like nitrogen, phosphorus, and potassium), microbial inoculants contain beneficial microorganisms that are intended to help plants access their own nutrients from the soil. Some of these products contain a single organism, others include a mixture of organisms, and some include proteins, enzymes, or other compounds intended to serve as food sources for the microorganisms.
Interest in these products is growing rapidly. According to Fortune Business Insights, the global microbial inoculant market was valued at $1.34 billion in 2018 and is projected to reach $3.15 billion by the end of 2026. Seed treatments of rhizobia and other nitrogen-fixing microorganisms accounted for 82.7% of this market.
The use of rhizobia bacteria to enhance nitrogen fixation by legumes (the Fabacae family, including peas, beans, alfalfa, clovers, and vetch) has been thoroughly studied by researchers since 1896, and rhizobia inoculum has been widely used to enhance nitrogen fixation since the 1950s. In contrast, there has been far less research on other microbial inoculants, especially under field conditions. Controlled studies in greenhouses have shown benefits such as enhanced nutrient uptake, resistance to drought and saline conditions, and protection against pest and disease infestations. Unfortunately, these benefits are not always seen in the field, under real-world conditions. This publication explains how microbial inoculants work, reviews current (in 2020) scientific literature and studies, explains several possible factors that can limit their effectiveness, and offers practical advice about buying and using these products.
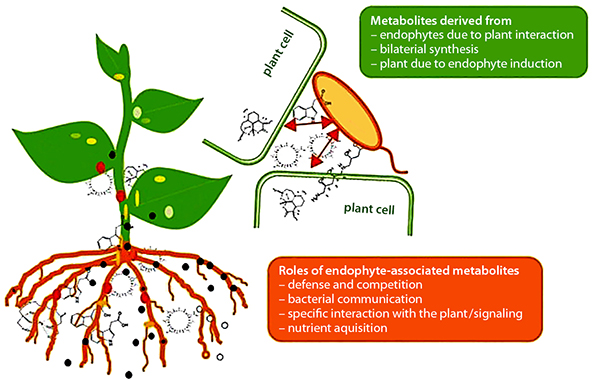
Plant-beneficial activities of endophytes. Source: Brader et al., 2014
Overview of Plant-Beneficial Microorganisms
Plant roots provide an excellent habitat for soil organisms. Osmosis and root pressure pull soil nutrients towards plant roots, making the root zone a nutrient-rich area. At the same time, plant roots exude many organic chemicals that serve as food sources for microorganisms.
Plant-beneficial microorganisms living in the rhizosphere (the area close to plant roots) include decomposers and bacteria involved in nitrogen transformations. Other microorganisms, called endophytes, live inside or between plant cells: protected from predators, getting most or all of their food from plants, and directly involved in plant growth processes. Still other microorganisms, like mycorrhizae, live partially within plant cells and partially within the rhizosphere.
Rhizobia
Rhizobia are a genus of rhizobacteria: bacteria that live in the rhizosphere. They live in large colonies in nodules on the roots of legumes, forming a symbiotic (mutually beneficial) relationship with their plant hosts. Rhizobia take nitrogen from the air (N2) and transform it into ammonia (NH4), which plants can use to form proteins and other biomolecules needed for growth. In return, the plant provides a place to live on its roots, as well as carbohydrates that the rhizobia use as energy.
To promote the formation of nodules and also nitrogen fixation, rhizobia inoculum in a powder form is mixed with peat or biochar, wetted, and applied to legume seeds immediately before planting. There are eight different rhizobia-legume cross inoculation groups, and it’s important to use the correct type of rhizobia for the legume being planted. (See the Further Resources section for guides to successful inoculation.)
Mycorrhizae
Mycorrhizae are fungi that form symbiotic relationships with almost 80% of all land plants. The mycorrhizae most important for agriculture are the arbuscular mycorrhizae (sometimes seen abbreviated as “AM”). Mycorrhizae begin their life within the cells of plant roots, where they extract carbohydrate energy and produce hyphae, thread-like structures that grow through the soil. Since these hyphae are considerably thinner than plant roots, they can grow more easily through soil, obtaining hard-to-reach phosphorus and water that are transported to plant roots.
As hyphae grow through and around soil particles, they bind soil particles into aggregates: clumps of soil mineral and organic matter that are key to soil nutrient and water-holding capacity and also enhance soil tilth or friability, decreasing soil compaction.
![]() Rhizobium bacteria. Source: McMahon, 2016 |
![]() Mycorrhizae. Source: Karas, no date |
![]() Common mycorrhizal networks. Source: Jansa et al., 2013 |
![]() Plant growth-promoting rhizobacteria. Source: Kumar and Dubey, 2012 |
Among their other benefits, mycorrhizae can produce chemicals that make iron and other micronutrients more available (Saha et al., 2015), make plants more resistant to saline conditions (Vurukonda et al., 2016), or produce phytohormones that signal the plant to create chemicals hindering the growth of pests and diseases (Hohmann and Messmer, 2017). Mycorrhizae can also connect with the roots of other nearby plants, including plants of different species. These common mycorrhizal networks can transfer nutrients, protective substances, and signals among plants—helping neighboring plants resist infestations from pests, diseases, or invasive plants. Some studies have shown that these mycorrhizal networks may be partially responsible for enhanced yields of multi-cropping systems (Walder et al., 2012).
Plant Growth-Promoting Rhizobacteria (PGPR)
Plant growth-promoting rhizobacteria (PGPR) are rhizosphere bacteria that (as the name implies) stimulate plant growth. Some PGPR protect against pathogens, stimulate production of growth regulators, or enhance a plant’s ability to detoxify poisons. Others enhance the availability of nutrients by fixing nitrogen, solubilizing phosphorus and potassium from soil minerals (Khan et al., 2007), or facilitating uptake of iron (Dobbelaere and Okon, 2007). PGPR can also work with mycorrhizae during droughts to enhance nodule formation on legume roots and nitrogen fixation by rhizobia (Pandey et al., 2016).
Table 1. Plant-Beneficial Rhizobacteria Species and Their Benefits
Nitrogen Fixation | Enhance nodules | Enhance | Phosphours Solubilization | > K uptake | Salinity Drought Stress | Disease Suppression | Plant & Root Growth | |
Agrobacterium | x | x | x | |||||
Arthrobacter | x | |||||||
Aspergillus | x | |||||||
Azoarcus | x | |||||||
Azotobacter | x | x | x | x | ||||
Azospirillum | x | x | x | |||||
Bacillus | x | x | x | x | x | x | x | x |
Burkholderia | x | x | x | |||||
Diazotrophicus | x | |||||||
Enterobacter | x | x | x | x | ||||
Herbaspirillum | x | |||||||
Klebsiella | x | x | x | |||||
Pseudomonas | x | x | x | x | x | x | x | |
Rhizobium | x | x | x | x | x | |||
Rhizopus | x | |||||||
Serratia | x | x | x | |||||
Trichoderma | x | x |
Sources: Mabrouk et al., 2018; Gouda et al. 2018; Tabassum et al., 2017; Bhardwaj et al., 2014
Like mycorrhizae, PGPR can produce phytohormones, improving tolerance for salinity, drought, and extreme temperatures (Vurukonda et al., 2016). One group of PGPR produces antibiotics and other chemicals that inhibit the growth of pathogens or their ability to enter and damage plant cells. PGPR can also stimulate protective responses to pest or disease infestation, such as exuding exopolysaccharides (Vurukonda et al., 2016).
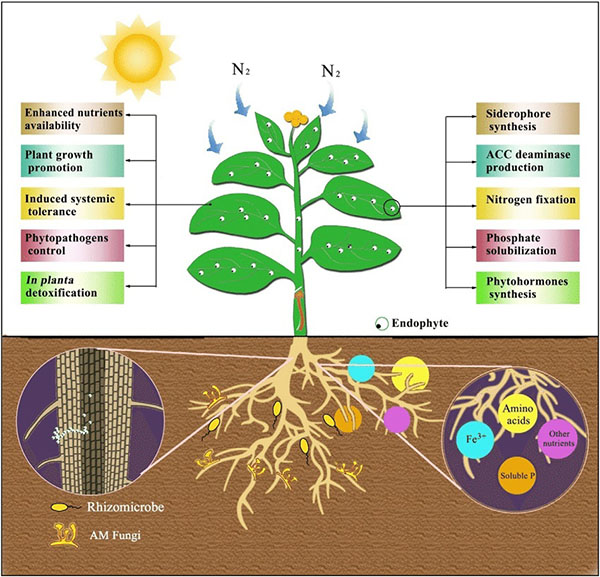
Benefits provided by endophytes. Source: Feng et al., 2017
Endophytes
As noted earlier, endophytes live inside or between plant cells—entering plant tissues through leaves, stems, or roots. Some PGPR are able to move from the rhizosphere into root tissue and become endophytes. Like PGPR, endophytes can stimulate plant stem and root growth. They can also protect plants against growth inhibition due to salinity, drought, flooding, pest and disease attack, presence of heavy metal or organic contaminants, or temperature (Glick, 2015). Endophytes have also been shown to enhance plant competitiveness against weeds (Moura et al., 2020) and to form phytochemicals in medicinal plants. While rhizobia are an example of endophytes, we discussed them separately above because of their widespread use and importance, as well as because these beneficial bacteria were known and promoted for use much earlier than other endophytes.
Plant-Beneficial Microorganism Interactions
Interactions among PGPR, mycorrhizae, and endophytes drive many important plant growth processes. For example, mycorrhizae hyphae, which typically only live five or six days, provide a source of food for PGPR (Staddon et al., 2003). In turn, substances produced by PGPR can affect root cell growth to facilitate the formation of mycorrhizal symbiosis (Azcón et al., 2010). PGPR, mycorrhizae, and endophytes often work together to enhance plant uptake of mineral nutrients, stimulate plant root growth, reduce drought stress, or produce substances that protect plants from pest and disease infestations. These interactions also enhance the nitrogen-fixing function of rhizobia by providing the rhizobia with phosphorous and protecting the nodules from stress conditions (Staudinger et al., 2016).
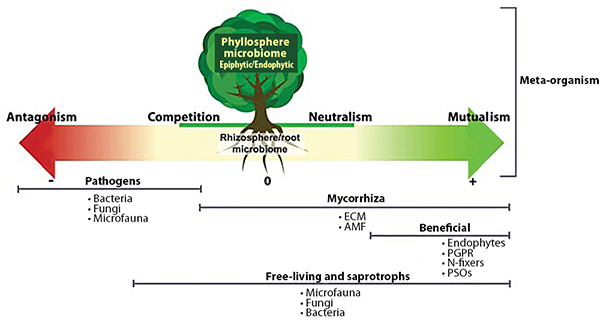
Soil microorganisms work together to provide plant benefits. Source: Quiza et al., 2015
Reasons Why Microbial Inoculants May Not Work
Soil is home to a vast ecosystem of soil organisms. Attempting to change that ecosystem by adding a relatively small amount of powder or solution containing new organisms—while sometimes dramatically effective—is also inherently problematic and unpredictable. Many factors can prevent microbial inoculants from working. These include competition with existing organisms, compatibility problems, inadequate or inappropriate diversity, unfavorable environmental conditions, unfavorable land management practices, and production factors that limit an inoculant’s effectiveness. Let’s look at each of these in more detail.
In-Field Competition
Recommended application rates for most microbial inoculant products are extremely small. If the added microorganisms are to provide any benefit, they need to grow, reproduce, and outcompete the vastly greater numbers of microorganisms already in the field for access to plant roots and other nutrient resources. For the introduced microorganisms to persist into the next growing season, they also need to benefit the plant sufficiently to preferentially obtain nutrients and habitat (Verbruggen et al., 2013).
Microorganism/Plant Compatibility
A microorganism may be parasitic or pathogenic to a particular plant species (Jones and Smith, 2004), extracting lots of plant carbohydrates while providing few, if any, benefits. In order to be effective, a beneficial microorganism must be compatible with the plant population, soil conditions, and existing soil microbial populations: providing substantial benefits while efficiently using (and not draining) the plant’s carbohydrates.
Lack of Diversity
A given soil may lack the necessary plant and microbial biodiversity to respond to a microbial inoculant. Different plant species exude different chemicals, release different nutrients back to the soil upon decomposition, and provide different types of microbial habitats within their rhizosphere. This diversity of physical and chemical conditions provided by plant diversity supports a diversity of microorganisms. Working together, a diversity of microorganisms provides many functions or services back to plants. For example, bacteria involved in nitrogen fixation, phosphorus solubilization, organic matter decomposition, and drought protection can all work together to provide nutrient cycling and continued plant growth under stress conditions.
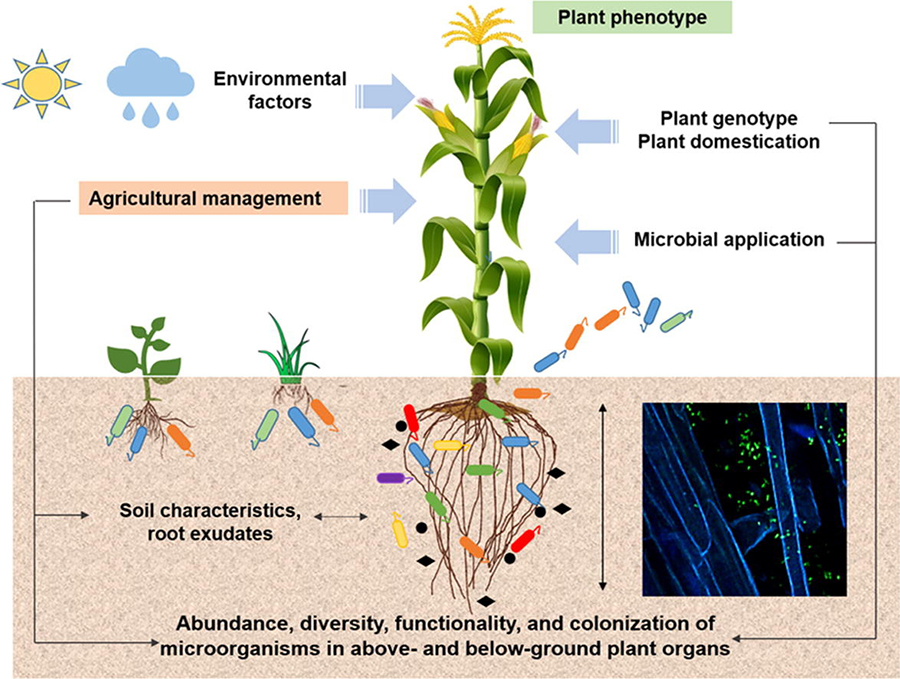
Environmental factors affecting plant benefits from soil microorganisms. Source: Compant et al., 2019
Environmental Conditions
Climate conditions, especially temperature, can limit the growth and beneficial properties of plant-beneficial microbes. For example, high temperatures can alter the release of metabolites from plant roots, favoring some microorganism strains over others. Drought and soil salinity inhibit plant growth and the formation of rhizobium nodules. However, certain PGPR can reduce these negative impacts. Soil conditions, such as aggregation, aeration, and moisture, will also favor some microorganisms over others.
Land Management Practices
Modern agriculture creates conditions that are very different from those under which most plant-microorganism relationships evolved, sometimes creating an unhospitable environment. Most crop plants are not native to the location where they are now grown and, due to plant breeding, most crops have characteristics that are different from native or heirloom varieties.
Beneficial microorganisms are also affected by farm inputs—especially manures, fertilizers, and pesticides. Symbiotic relationships between soil microbes and plants evolved under stress conditions, with limited plant access to soil nutrients. When nutrients are abundantly available from manure or synthetic fertilizers, a plant obtains limited benefits from developing associations with microorganisms and may not produce exudates to support the growth of these now-unnecessary microorganisms (Djuuna, 2014). Chemicals in pesticides can also kill or disrupt the growth of microorganisms.
Microbial Inoculant Production Method
Not all varieties of mycorrhizae or PGPR are readily made under laboratory or large-scale production conditions. For example, the mycorrhizae and PGPR that provide the greatest benefits to plants growing under stress conditions are typically slower-growing varieties, whereas rapidly-growing varieties are appealing to manufacturers because they are more cost-effective to produce. Growing conditions in commercial production are also usually very different from field conditions. In the greenhouse or in soilless culture, mycorrhizae are produced on a single host plant, while bacteria are produced in aerated vats of nutrients and minerals. Such favorable growing conditions—better than typical field conditions—favor organisms that provide fewer benefits than those grown under more stressed conditions (Hohmann and Messmer, 2017).
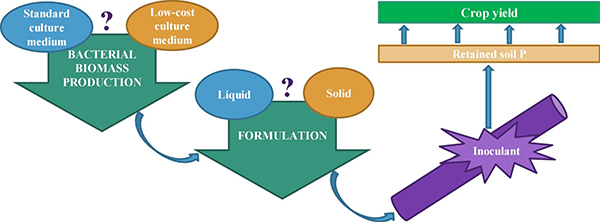
Commercial microbial inoculants need microorganisms, a material to carry and nurture them, and packing to enhance their viability. Source: Lobo et al., 2019
Overcoming Microbial Inoculant Limitations
Research has shown the following factors to be necessary for microbial inoculants to be effective:
- The diversity of organisms in microbial inoculants, and the nutrients added to them, should be consistent with natural ecological processes.
- Microbial inoculants should be produced under soil, crop production, and environmental conditions similar to those where these products will be used.
- Processing, packaging, customer handling, and use of microbial inoculants need to protect the viability of the microorganisms.
- Microbial inoculants must be applied to seeds or plant roots in a way that favors competition of the added microorganisms with resident soil organisms.
Let’s consider these one at a time:
Microbial and Nutrient Diversity
No microbial inoculant is effective in all ecosystems. Some products include a small number of microbial species and are designed to provide a specific function. Other products contain diverse species and are designed to provide multiple benefits. Moreover, some products also contain enzymes and micronutrients, intended to stimulate initial growth of the microorganisms. Before buying, study the label to determine the type and diversity of microorganisms in the product and whether any nutrients are provided.
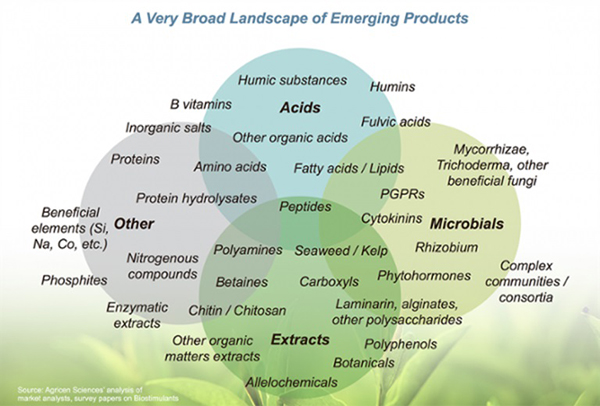
Microbial inoculants may contain nutrients and hormones. Source: Agricen, no date
Production Conditions
Ideally, a microbial inoculant should be produced under conditions closely resembling those where the product will be used. Most products commercially available in the United States are developed for a mass market. In developing countries, when products are developed for specific, localized conditions, inocula have proven effective (Gouda et al., 2018). While currently cost-prohibitive for commercial production, genetic sequencing can be used to select microorganisms with specific beneficial or environmental traits (Paterson et al., 2017) and to identify processes influencing establishment success and persistence of inoculants in the soil under field conditions (Kokkoris et al., 2019).
The Rodale Institute guide How to Inoculate Arbuscular Mycorrhizal Fungi on the Farm, describes a method for creating a “diverse group of locally-adapted mycorrhizal fungi that can be used to boost a farm’s native population” (Lohman et al., 2010). Steps include:
- Select a host plant and grow it to seedling stage in vermiculite or seed starter. (Don’t use Brassicaceae species as host plants, since most plants in that family don’t form mycorrhizal associations.)
- Transplant the seedlings into pots filled with 1:3 soil:sand mixture, using sterilized field soil or soil from a natural area or field that has not been used within two years to grow the crop you are planning to inoculate.
- Make a dilute compost mix by combining compost (yard waste or dairy manure) with a nutrient-poor substrate such as vermiculite, perlite, or peat in 7-gallon bags. For yard-clipping compost, the article suggests a ratio of 1:4 (compost:vermiculate) on a volume basis.
- Collect soil from the top five inches of soil at various locations throughout a natural area. Add about a quart of this local soil to each of the 7-gallon bags and mix. Transfer about five seedlings into each 7-gallon bag. Weed and water. Mycorrhizhae will proliferate as the plants grow.
- To harvest mycorrhizal inoculum after the plants have grown, shake loose compost/vermiculite mix from the root ball. Then cut the root segments into short pieces (less than ½ inch) and add these to the compost/vermiculite mix that you shook loose.
This method requires that you have access to natural soil with mycorrhizal fungi present. Another approach is simply to dig up plants from local healthy ecosystems (assumed to have plenty of mycorrhizae), and harvest mycorrhizal inoculum by shaking soil loose from the roots and cutting the roots up into small pieces.
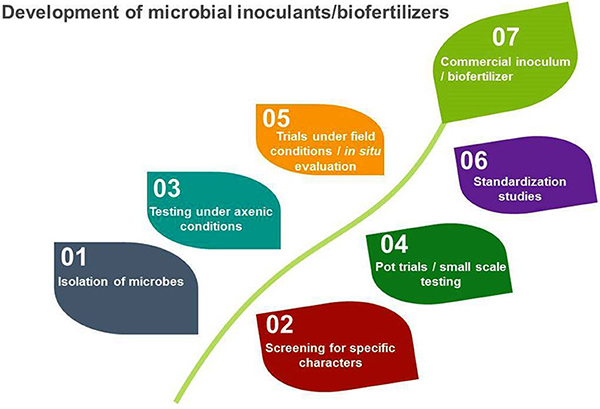
The microbial inoculant production process. Source: Ahmad et al., 2018
Packaging, Handling, and Application
Mycorrhizal and PGPR inoculants are sold either as powders or liquid solutions. Liquid formulations are less expensive to make but have shorter storage times, losing viability after only about six months. Solid formulations are mixed with clay, peat, biochar, and various agricultural by-products such as cassava starch, sugarcane bagasse, or talc. Encasing microorganisms in microcapsules made of plant-based polymers or resins has been shown to enhance shelf life, while allowing slow release of microorganisms and providing protection from predation during the establishment stage (Hernández-Montiel et al., 2017). Another method that has shown effectiveness is to mix microbial inoculants with biofilms and apply them to plant seeds. (Biofilms are substances exuded by soil organisms onto plant root or leaf surfaces.)
In general, seed application has proven to be the most effective method of applying mycorrhizal and PGPR inoculants to field crops, allowing beneficial microorganisms to grow with the germinating seed. Seed treatment involves mixing a solution or moist powder containing spores of the microorganisms with seeds just prior to planting.
Transplanted vegetables are best inoculated by dipping the roots into a solution or paste of the inoculum. Inoculum can also be added to the transplant soil hole when transplanting trees or potted plants. Microbial applications have been very effective in horticulture and tree nurseries, primarily due to the direct application of the inoculum to potted plants or tree seedlings.
Summary, Future Directions, and Recommendations
More than 30 years of greenhouse-based controlled experiments have demonstrated highly beneficial relationships among plants, mycorrhizae, PGPR, and endophytes. However, these benefits have not been consistently reported for the use of commercial products under field conditions.
Standard sustainable agricultural practices will likely enhance the benefits provided by microbial inoculants. These practices include:
- Eliminating pesticides that hinder microbial growth
- Avoiding tillage and other soil disturbance
- Not over-applying fertilizers or manure
- Enhancing plant biodiversity
- Ensuring that a crop is growing or has a root in the ground throughout the year
Inoculants can be produced on-farm using soil from organic or limited input fields as the inoculum source. Alternatively, commercial inoculums can be combined with local soil to produce locally adapted inoculum.
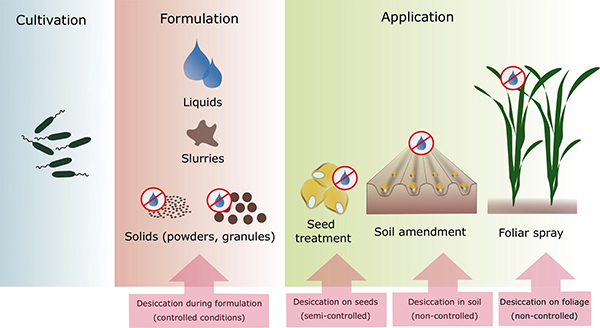
An eff ective microbial inoculant needs the right microorganisms, production conditions, packaging, and substances to enhance longevity. Source: Berniger et al., 2018
Researchers are currently (in 2020) studying the genetics of particular microorganism species and isolating those organisms to produce inoculants (Agnolucci et al., 2019). Recent research (Rouphael and Colla, 2018) demonstrates synergistic benefits when microbial inoculants include a combination of PGPR, mycorrhizae, and humic acids (a complex form of organic matter). Wild plants that are able to survive harsh environmental conditions are also being explored as a source for endophytes (Afzal et al., 2019).
Since the most effective inoculants are developed for specific plants and environmental conditions, effective commercial inoculants will likely be developed sooner for high-value crops (such as ornamentals and vegetables) than for field crops. In the meantime, land management practices that promote soil health and microbial populations are likely the most cost-effective method for enhancing rhizosphere microbial communities.
As this publication is going to press (in 2020), the European Union is about to establish a Fertilizing Products Regulation: providing a claims-based definition of “biostimulants” or microbial inoculants. To gain access to European markets, a manufacturer will need to produce data showing that the product reliably provides the benefits claimed (Ricci et al., 2019). While similar legislation has not yet been proposed in the United States, these EU standards may eventually influence U.S. legislation.
Before using any commercial inoculant, read the label carefully to determine the type of organisms and supplementary nutrients or enzymes it contains. Also, check the production date to ensure that the inoculum is still viable. Keep it in a cool, dry location to slow loss of viability. When using the product, make sure it adheres to the seeds and that the seeds are planted immediately, so the inoculant doesn’t dry out. To enhance the benefits of any added inoculum, follow sustainable agricultural practices such as minimizing input use, planting a diverse mix of cover crops, and avoiding tillage.
References
Afzal, Imran, Z.K. Shinwari, S. Sikandar, and S. Shahzad. 2019. Plant-beneficial endophytic bacteria: Mechanisms, diversity, host range and genetic determinants. Microbiological Reseawrch. Vol. 221. p. 36-49.
Agnolucci, Monica, L. Avio, A. Pepe, A. Turrini, C. Cristani, P. Bonini, V. Cirino, F. Colosimo, M. Ruzzi, and M. Giovannetti. 2019. Bacteria associated with a commercial mycorrhizal inoculum: community composition and multifunctional activity as assessed by illumina sequencing and culture-dependent tools. Frontiers in Plant Science. Vol. 9, Article 1956.
Agricen. No date. Agricultural Biostimulants.
Ahmad, Maqshoof, L. Pataczek, T.H. Hilger, Z.A. Zahir, A. Hussain, F. Rasche, R. Schafleitner, and S. Solberg. 2018. Perspectives of Microbial Inoculation for Sustainable Development and Environmental Management. Frontiers in Microbiology. Vol. 9.
Azcón, Rosario, J.M. Barea, and C. Azcón-Aguilar. 2010. Mycorrhizosphere Interactions for Legume Improvement. Microbes for Legume Improvement. Springer, Vienna. p. 199-225.
Berninger, Teresa, Óscar González López, Ana Bejarano, Claudia Preininger, Angela Sessitsch. 2018. Maintenance and assessment of cell viability in formulation of non‐sporulating bacterial inoculants. Microbial Biotechnology. Vol. 11, No. 2. p. 277-301.
Bhardwaj, D., M.W. Ansari, R.K. Sahoo, and N. Tuteja. 2014. Biofertilizers function as key player in sustainable agriculture by improving soil fertility, plant tolerance and crop productivity. Microbial Cell Factories. Vol. 13, No. 66.
Brader, Günter, S. Compant, B. Mitter, F. Trognitz, and A. Sessitsch. 2014. Metabolic potential of endophytic bacteria. Current Opinion in Biotechnology. Vol. 27. p. 30–37.
Compant, Stephane, A. Samad, H. Faist, and A. Sessitsch. 2019. A review on the plant microbiome: Ecology, functions, and emerging trends in microbial application. Journal of Advanced Research. Vol. 19. p. 29-37.
Djuuna, Irnanda A.F. 2014. Arbuscular Mycorrhizal Colonization and Agricultural Land Use History. In: Solaiman Z., L. Abbott, and A. Varma (eds). Mycorrhizal Fungi: Use in Sustainable Agriculture and Land Restoration. Soil Biology. Vol. 41. Springer, Berlin, Heidelberg.
Dobbelaere, S., and Y. Okon. 2007. The Plant Growth-Promoting Effect and Plant Responses. Associative and Endophytic Nitrogen-fixing Bacteria and Cyanobacterial Associations. Nitrogen Fixation: Origins, Applications, and Research Progress. Vol. 5. Springer, Dordrecht.
Feng, Nai-Xian, J. Yu, Jiao, H.M. Zhao, Y.T. Cheng, C.H. Mo, Q.Y. Cai, Y.W. Li, H. Li, M. Wong. 2017. Efficient phytoremediation of organic contaminants in soils using plant-endophyte partnerships. Science of The Total Environment. Vol. 583. p. 352-368.
Glick, B.R. 2015. Beneficial Plant-Bacterial Interactions. Springer, Heidelberg.
Gouda, Sushanto, R.G. Kerry, G. Das, S. Paramithiotis, H.S. Shin, J.K. Patra. 2018. Revitalization of plant growth-promoting rhizobacteria for sustainable development in agriculture. Microbiological Research. Vol. 206. p. 131-140.
Hernández-Montiel, Luis G., C.J. Chiquito Contreras, B.M. Amador, L.V. Hernández, E.E. Quiñones Aguilar, and R.G. Chiquito Contreras. 2017. Efficiency of two inoculation methods of Pseudomonas putida on growth and yield of tomato plants. Journal of Soil Science and Plant Nutrition. Vol. 17, No. 4. p. 1003-1012.
Hohmann, P. and M.M. Messmer. 2017. Breeding for mycorrhizal symbiosis: focus on disease resistance. Euphytica. Vol. 213. p. 113.
Janczak, Katarzyna, K. Hrynkiewicz, Z. Znajewska, and G. Dąbrowska. 2018. Use of rhizosphere microorganisms in the biodegradation of PLA and PET polymers in compost soil. International Biodeterioration & Biodegradation. Vol. 130. p. 65-75.
Jansa, Jan, P. Bukovska, and M. Gryndler. 2013. Mycorrhizal hyphae as ecological niche for highly specialized hypersymbionts—or just soil free riders? Frontiers in Plant Science. Vol 4. p. 134.
Jones, Melanie D., and S.E. Smith. 2004. Exploring functional definitions of mycorrhizas: Are mycorrhizas always mutualisms? Canadian Journal of Botany. Vol. 82. p. 1089–1109.
Karas, Sheryl. No date. How the Fungus Might Save Us. Center for Regenerative Agriculture, University of California,
Chico.
Khan, M.S., A. Zaidi, and P.A. Wani. 2007. Role of phosphate-solubilizing microorganisms in sustainable agriculture — A review. Agronomy and Sustainable Development. Vol. 27. p. 29-43.
Kokkoris, Vasilis, Y. Li, C. Hamel, K. Hanson, and M. Hart. 2019. Site specificity in establishment of a commercial arbuscular mycorrhizal fungal inoculant. Science of the Total Environment. Vol. 660. p. 1135–1143.
Kumar, Pankaj and R.C. Dubey. 2012. Plant growth-promoting rhizobacteria for biocontrol of phytopathogens and yield enhancement of Phaseolus Vulgaris L. Journal of Current Perspectives in Applied Microbiology. p. 6-38.
Lobo, C.B., M.S. Juarez Tomas, E. Viruel., M.A. Ferrero, and M.E. Lucca. 2019. Development of low-cost formulations of plant growth-promoting bacteria to be used as inoculants in beneficial agricultural technologies. Microbiological Research. Vol. 219. p. 12-25.
Lohman, M., C. Ziegler- Ulsh, and D. Douds. 2010. How to innoculate arbuscular mycorrhizal fungi on the farm, Part 1. Rodale Institute.
McMahon, Julie. 2016. Nitrogen-Fixing Microbes in Legumes. University of Illinois College of Liberal Arts and Sciences.
Mabrouk, Yassine, Imen Hemissi, Issam Ben Salem, Sonia Mejri, Mouldi Saidi, and Omrane Belhadj. 2016. Potential of Rhizobia in Improving Nitrogen Fixation and Yields of Legumes. p. 107-119. In: Everlon Rigobelo (ed.). Symbiosis.
Meena, Vijay Singh, S.K. Meena, J.P. Verma, A. Kumar, A. Aeron, P.K. Mishra, J.K. Bisht, A. Pattanayak, M. Naveed, and M.L. Dotaniya. 2019. Plant-beneficial rhizospheric microorganism (PBRM) strategies to improve nutrients use efficiency: A review. Ecological Engineering. Vol. 107. p. 8-32.
Mishra, R., and V. Sarma. 2017. Mycoremediation of Heavy Metal and Hydrocarbon Pollutants by Endophytic Fungi. In: Prasad R. (ed.). Mycoremediation and Environmental Sustainability. Fungal Biology. Springer, Cham.
Moura, Mariana S., J.W.F. Lacerda, K.A. Siqueira, B.S. Bellete, P.T. Sousa Jr, E.L. Dall´Óglio, Marcos A. Soares, L.C.C. Vieira, and O.M. Sampaio. 2020. Endophytic fungal extracts: evaluation as photosynthesis and weed growth inhibitors. Journal of Environmental Science and Health, Part B. Vol. 55, No. 5. p. 470-476.
Paterson, Julia, G. Jahanshah, Y. Li, Q. Wang, S. Mehnaz, and H. Gross. 2017. The contribution of genome mining strategies to the understanding of active principles of PGPR strains. FEMS Microbiology Ecology. Vol. 93, No. 3. p. fiw249.
Pandey, Sonali, A. Verma, and D. Chakraborty. 2016. Recent Trends in Biofertilizers. 1st Edition. Bikas R. Pati, Santi M. Mandal (eds.). I.K. International Publishing House. p.116-140.
Quiza, Liliana, M. St-Arnaud, and E. Yergeau. 2015. Harnessing phytomicrobiome signaling for rhizosphere microbiome engineering. Frontiers in Plant Science. July 14.
Rouphael, Youssef, and G. Colla. 2018. Synergistic biostimulatory action: designing the next generation of plant biostimulants for sustainable agriculture. Vol. 9, Article 1655.
Ricci, Manuele, L. Tilbury, B. Daridon, and K. Sukalac. 2019. General Principles to Justify Plant Biostimulant Claims. Frontiers in Plant Science. Volume 10, Article 494.
Saha, M., S. Sarkar, B. Sarkar, B. Sharma, S. Bhattacharjee, and P. Tribedi. 2015. Microbial siderophores and their potential applications: a review. Environ Science and Pollution Research. March 12. Vol. 23. p. 3984–3999.
Staddon, Philip L., C.B. Ramsey, N. Ostle, P. Ineson, and A.H. Fitter. 2003. Rapid Turnover of Hyphae of Mycorrhizal Fungi Determined by AMS Microanalysis of 14C. Science. Vol. 16. p. 1138-1140.
Staudinger, Christiana, V. Mehmeti-Tershania, E. Gil-Quintan, E.M. Gonzalez, F. Hofhansl, G. Bachmann, and S. Wienkoop. 2016. Evidence for a rhizobia-induced drought stress response strategy in Medicago truncatula. Journal of Proteomics. Vol. 136. p. 202-213.
Tabassum B., A. Khan, M. Tariq, M. Ramzan, M.S. Iqbal Khan, N. Shahid, and K. Aaliya. 2017. Bottlenecks in commercialisation and future prospects of PGPR. Applied Soil Ecology. Vol. 121. p. 102-117.
Verbruggen, Erik, Marcel G.A. van der Heijden, Matthias C. Rillig, and E. Toby Kiers. 2013. Mycorrhizal fungal establishment in agricultural soils: factors determining inoculation success. New Phytologist. Vol. 197, No. 4. p. 1104-1109.
Vurukonda, S.S.K. Prasad, S.Vardharajula, M. Shrivastava, and A. Skz. 2016. Enhancement of drought stress tolerance in crops by plant growth-promoting rhizobacteria. Microbiological Research. Vol. 184. p. 13-24.
Walder, Florian, H. Niemann, M. Natarajan, M.F. Lehmann, T. Boller, and A. Wiemken. 2012. Plant Physiology. June. p. 789-797.
Further Resources
Legume Seed Inoculation. USDA Natural Resource Conservation Service (NRCS). Technical Note TX-PM-15-01.
This document from NRCS guides producers through the process of selecting and inoculating legume cover crops. It also discusses considerations for storage of inoculant and checking for effective inoculation.
How to Inoculate Arbuscular Mycorrhizal Fungi on the Farm, Part 1. 2010. By Rodale Institute, Kutztown, PA.
This resource provides an overview of mycorrhizal fungi’s role in a sustainable agroecosystem and then goes into detail on how to produce native mycorrhizal inoculant on-farm.
A Guide to Troubleshooting Nodulation Failure in Leguminous Cover Crops. 2019. By University of Texas Rio Grande Valley.
This guide walks a producer through a decision tree to assist in determining the cause of nodulation failure in a legume cover crop.
Microbial Inoculants
By Barbara Bellows, Texas Institute for Applied Environmental Research; Mike Morris, NCAT; and Colin Mitchell, NCAT
Published September 2020
©NCAT
IP 604
Slot 630
This publication is produced by the National Center for Appropriate Technology through the ATTRA Sustainable Agriculture program, under a cooperative agreement with USDA Rural Development. ATTRA.NCAT.ORG.