Biochar and Sustainable Agriculture
By Jeff Schahczenski, NCAT Agricultural and Natural Resource Economist
Biochar is a potentially valuable soil amendment produced from biomass, through pyrolysis. Biochar improves soil aggregation, enhances nutrient- and water-holding capacity, provides habitat for soil organisms, modulates microbial activity and biodiversity, and may stabilize soil organic carbon (SOC). Pyrolysis also yields biofuels in the form of combustible gases or oils. Biochar is thought to mitigate climate change by providing both renewable energy and a soil amendment that may significantly enhance net soil carbon (C) sequestration. However, additional research is needed before biochar applications to fields and grazing lands can be validated as a carbon offset and a potential source of income for farmers and ranchers.
This publication reviews the current research and issues surrounding the production and use of this biomass energy technology and explores how biochar can contribute to sustainable agriculture. It focuses on the use of sustainable biochar with the intention of adding it to crop production systems and possible benefits for climate change mitigation.
Introduction
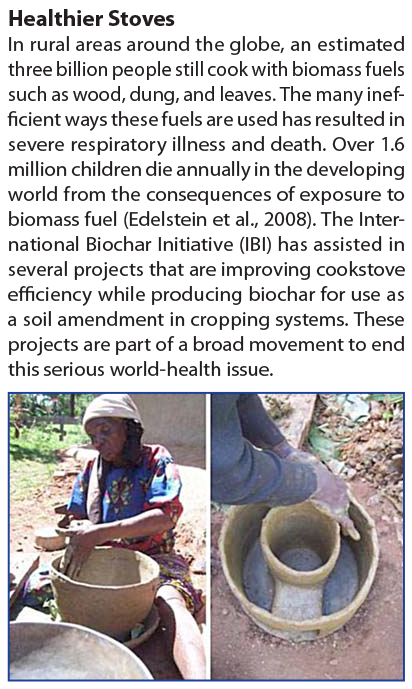
Biochar stove in Kenya: Making a biochar stove in Kenya. Photos: Courtesy of International Biochar
Initiative
The origins of the study of biochar lie not in modern agricultural practices but in the exploration and archeological study of early human settlement and soils. These early studies of soils being enriched from what appears to be the deliberate mixing of burned biomass in soils around human settlements helped spark more recent interest in biochar. These deposits of enriched soils, known as terra preta in the Amazon region of South America, have developed into a full field of scientific study of their own (Lehmann et al., 2004). The terra preta soils stand in marked contrast to the adjacent highly weathered, low-fertility, red, tropical-forest soils (Oxisols) from which they were derived, and their fertility appears to result from combined inputs of char plus other organic materials such as food scraps and human excrement over a time span of centuries (Wilson, 2014).
Naturally occurring wildfires also create char, which can lead to the development of dark, highly fertile soils, such as the Mollisols of the Midwestern United States and the Chernozems of the Russian steppe. In these regions, periodic prairie fires consume dry grass tops completely, but leave black carbon (i.e., biochar) at and near the soil surface. The black carbon thus accumulated over millennia is thought to comprise some 40 to 50% of total soil organic carbon (SOC) in these soils.
More current studies of the biochar production process are focused on its role in a growing demand for biomass-based energy sources whose use in lieu of fossil fuels can mitigate greenhouse-gas emissions and slow climate change. For more information about bioenergy, see the ATTRA publication An Introduction to Bioenergy: Feedstocks, Processes and Products. In addition, biochar has the potential to enhance soil quality and soil carbon se
questration. For more information about carbon sequestration, see the ATTRA publication Agriculture, Climate Change and Carbon Sequestration. A secondary source of interest in biomass pyrolysis comes from the growing need to develop low-cost and healthier (less polluting) biomass-fueled stove technology.
What is Biochar?
The definition of biochar is more about its creation and intended application than its composition. Biochar is created through an energy-conversion process called pyrolysis. Pyrolysis is the combustion of biomass in the complete or near absence of oxygen. Pyrolysis of biomass produces biochar, oils, and gases. The amount of these outputs produced depends on processing conditions. Biochar can be produced from a variety of biomass feedstocks (wood, manure, grasses, and crop residues). The oils and gases from pyrolysis can be used for energy production. The biochar and energy created during pyrolysis may provide both a renewable energy source and a valuable coproduct for soil improvement. Together, these products have the potential for a “negative” carbon footprint, as discussed later in this publication.
However, not all biochars are created equal. The efficiency and effectiveness of biochar creation and use can vary, and the specific biomass sources used can affect the characterization and suitability of the biochar as a soil amendment that enhances crop and forest productivity (McLaughlin et al., 2009).
Complex ongoing research is striving for a more uniform and standard biochar that will limit potential environmental problems associated with biochar production and application to soils. Standards for low-environmental-impact processes may make it possible for people who buy biochar to ensure that their use of the soil amendment does not entail a large negative environmental footprint. Product labels can include important measures of product qualities such as C, N, P, and K content, trace elements and heavy metals, moisture and ash content, pH and liming equivalent, CEC, and degree of aging (in months or years, similar to aged cheese). Technical hurdles related to measuring metals, alkalinity, and some other parameters may remain.
Some of the attributes of biochars can go beyond just physical characteristics to issues of whether the feedstock was from a sustainably harvested and renewable source, if its production reduced greenhouse gas emissions, and if the biochar can improve soil quality in a reliable way. The International Biochar Initiative (IBI) has developed a voluntary biochar standard and certification program (IBI, 2009; 2015b).
Importance to Farmers and Ranchers
Increased Fertility
Farmers and ranchers may have an interest in biochar as a soil amendment that can enhance fertility and reduce the need for other fertilizers that entail both direct and environmental costs. Biochar also shows considerable potential to improve soil physical properties, including aggregate stability, porosity and moisture infiltration, and water-holding capacity (Blanco-Canqui, 2017; Sandhu and Kumar, 2017). However, practical issues of cost, how much to apply, availability, and possible risks with application are yet to be fully explored, even though research is expanding rapidly. The book Biochar for Environmental Management: Science, Technology and Implementation, 2nd Edition, edited by Johannes Lehmann and Stephen Joseph, has some of the best current (2015) information available. Scientists still don’t have a full understanding of how biochar provides fertility for crops, but the following provides a good summary of what research has suggested to date:
- Biochars have variable and often limited plant-nutrient content per se. Pyrolysis removes at least half of the N content of the original feedstock (North, 2015), yet may concentrate base cations such as potassium (K) and calcium (Ca), depending on ash content of the biochar product. For example a Swiss Biochar product contained 0.8% potash (K2O) (Schmidt and Niggli, 2015), which would deliver 160 pounds of K2O per acre in a 10-ton-per-acre application. Biochars made with manure generally have higher nutrient content than plant-based biochars.
- Biochar enhances soil fertility primarily by providing cation exchange capacity (CEC); reducing leaching losses of nitrate, phosphate, and other anion nutrients, improving soil structure and moisture-holding capacity, and enhancing soil biology (Blanco-Canqui, 2017; Petersen-Rockney, 2015; Wilson, 2014).
- The enhanced availability of nutrients to crops made possible by biochar is likely enhanced if the biochar is blended with compost, manure, or synthetic fertilizer before application. Biochar combined with synthetic nitrogen increased wheat yields in Oregon (Machado et al., 2017). Blending biochar in this way is often referred to as “charging” the biochar.
- Biochar does not work alone; it is most effective when used in conjunction with other organic practices and inputs such as cover cropping and compost. The high fertility and stable SOC content of Amazonian terra preta soils and prairie soils result from synergistic interactions among the black carbon inputs, living vegetation, other organic residues, and soil biota–not from the char alone (Wilson, 2014). Similarly, field applications of a mixture of biochar and dairy manure (total 4.5 tons per acre) improved soil water-holding capacity to a greater degree than either material alone, applied at the same rate (Sandhu and Kumar, 2017).
- The high surface area and pore structure of biochar provide a habitat for soil microorganisms including N-fixing bacteria, as well as beneficial fungi, which in turn can make some nutrients more available to crops (Petersen-Rockney, 2015).
- The efficacy of biochar in enhancing crop yields can depend on many factors: the quality of the biochar product itself (feedstock, pyrolysis temperature, procedure, time elapsed between manufacture and use), soil type and texture, existing soil condition (or soil health), and the crops grown. Benefits to soil physical properties (e.g., tilth, water holding capacity) are greatest in sandy soils (Blanco-Canqui, 2017). The largest positive yield responses to biochar tend to occur in acidic, low-fertility, or degraded soils (Kittredge, 2015), such as in the Amazon basin, where centuries of indigenous practices that included biochar built the terra preta.
- Biochar is often alkaline, with a significant liming effect related to its ash content. In cooperative trials with 144 European vegetable gardeners, vegetables in the crucifer, cucurbit, and umbel (carrot) families showed a 25 to 30% yield response to biochar at ~4.5 tons per acre, while yields of solanaceous (tomato, potato, eggplant) vegetables decreased ~15% and pea, bean, and lettuce yields were unaffected (Schmidt and Niggli, 2015). The first three plant families benefited from the alkalizing effect and K supplied by the biochar product used, whereas solanaceae prefer more acidic soils and were slightly harmed by the alkalinity of the product.
- Aging of biochar after production may be critical for efficacy (Wilson, 2014). Oxidative processes during biochar aging develop negative surface charges (= CEC), promote organo-mineral stabilization of soil organic carbon (SOC), and may enhance crop-yield response to biochar amendments (Mia et al., 2017). SOC and soil organic N increased with years after a 10-ton-per-acre application in several cropping systems (Aller et al., 2017).
- Research on whether biochar can provide improved nitrogen and phosphorus availability to crops is not definitive but is suggestive of a positive effect.
Moisture Retention
A few studies of biochar application on crops suggest that biochar shows differing results as far as enhancing soil moisture retention. This attribute of biochar may lessen the effects of drought on crop productivity in drought-prone areas. As noted above, this moisture-retention capacity is largely related to the high surface area and porosity of biochar. There is some controversy because the moisture-retention capacity is related to the feedstock that was used to produce the biochar, as well as the exact production process. These two factors can affect the pore and surface structure of the biochar. However, if climate change leads to even drier conditions in many agricultural production areas, biochar as a soil amendment may still have some positive effect on retaining soil moisture, even if it is variable from various feedstock sources (Lehmann and Joseph, 2015; Shafaqat et al., 2017).
Liming Effects
For acidic soils that require liming, there is growing evidence that biochar may improve soil pH balance (Collins, 2008). More biochar may need to be applied, relative to liming. However, the substitution of biochar for lime can likely provide for net carbon benefit compared to standard liming. It is not known how long the liming effect of biochar lasts in soil (Jeffery et al., 2015). Biochar seems to improve overall cation exchange capacity (CEC), which would also improve pH balancing and buffering. Biochar may provide a way to reduce acid toxicity in soils that is related to long-term synthetic fertilizer use and no-till cropping practice.
On-Farm and Community-Based Bioenergy Production
The process of making biochar has the potential to be scaled to a level that allows biochar and bioenergy to be produced on the farm or as a rural community economic-development project. Farms and ranches have the advantage of being close to several sources of biomass that would be appropriate for biochar production and use. A few demonstration and research projects in the United States are just beginning to examine biochar production but have so far been largely limited to forestry-based biochar and liquid fuel and electric bioenergy production. One recent study (2017) provided a farm-scale case study of biochar production and utilization in Washington State (Phillips et al., 2018). The study concluded that on-farm biochar production from farm-created waste biomass is physically possible for meeting crop demands for both power and biochar application. However, on-farm production only provided enough biochar annually to cover 6.3 to 11.8% of the land in production. Links to other biochar projects are provided at the end of this publication.
Economic Potential of Biochar
There are three sources of biochar economic potential for farmers and ranchers: as a soil amendment that could partially replace fertilizer; as a source of heat, bio-oil, and gases for farm and ranch use; and as a carbon offset in future cap-and-trade markets. For example, it is conceivable that a farm or ranch with significant renewable biomass sources available for harvest could covert that biomass to heat and liquid or gas fuel for machinery operation and also return the biochar back to the fields to enhance fertility and collect a carbon-offset payment. However, note that several economic, institutional, and regulatory questions need to be answered before such a project could be fully optimized.
Costs and Value of Biochar
What are the costs and values of biochar production? The answer to this question is still very much an open research issue. A good place to start is a study published by Washington State University, which estimates a wide range of costs for biochar and bio-oil production (Granatstein et al., 2009). As noted earlier, the creation of biochar results in not only char but also oils (bio-oil) and gases that have potential economic value. Depending on the scale of production, which ranges from mobile to stationary theoretical production units, the WSU study suggests a wide range in total costs of production for biochar. However, total estimated revenue from both biochar and bio-oil, subtracted from estimated total production costs, is negative for all four types of biochar facilities analyzed (Granatstein et al., 2009). Thus, biochar production appears to be unprofitable.
However, in this study, biochar’s price is based on a comparison of the relative energy value of biochar to coal and is estimated to be $114 per ton. The bio-oil is valued at $1.06 per gallon based on its comparison to heating oil. Consequently, biochar in this study does not appear to be produced economically unless either bio-oil or biochar can be sold for greater than these estimated prices.
More recently, biochar industry leaders have suggested that biochar is being sold in the United States at a price between $0.12 and $1.50 per pound, or $240 to $3,000 per ton (Biomass Magazine, 2017). The extreme range of price is attributed to the diversity of biochar markets, such as biochar used as an amendment to garden fertilizers, in mine remediation, and in research projects. Also, an increasing number of companies in the United States and worldwide are supplying biochar. There were an estimated 326 biochar companies worldwide in 2015, indicating commercial feasibility of biochar for some uses (IBI, 2015a).
A more recent study (2017) was done to estimate the economic value of biochar for carbon sequestration in the state of Massachusetts (Timmons, et al.). The study was based on forest biomass sources. Researchers estimate the agricultural value of biochar to be $57 per ton, but the cost of biochar production after subtracting this agricultural value ranges from $211 per ton to $304 per ton.
The study used actual information from five biochar production facilities at different scales of production currently operating in Massachusetts. The study also calculates the agricultural value of biochar to Massachusetts farmers based on crop-production studies that indicate an estimated 10% increase in the value of all agricultural production in Massachusetts.
However, since the focus of this study was on the carbon-sequestration economics of biochar, the value of biochar as an energy source was not evaluated. Nonetheless, for these five alternative biochar production methods, biochar would have to sell for much more than its $211 to $304 per ton estimated cost. Calculating the carbon-sequestration cost of biochar requires additional estimates of the carbon content of biochar and what percentage of the carbon in biochar sequestered in soil remains “recalcitrant” or stable. The cost of sequestration in this study is estimated to range from $83 to $119 per Mega-gram (Mg) of carbon dioxide (CO2). This estimated cost is important, as it implies a break-even price offering for CO2 mitigation in a potential cap-and-trade carbon market. Thus, a farmer or rancher could offset the cost of biochar by receiving carbon-credit payments from its application.
Overall, this study estimates that the potential of biochar to mitigate climate impacts and produce renewable biomass energy is modest. If all sustainably harvested biomass from Massachusetts forests were to be used for biochar production, an estimated 71 biochar processing facilities would be required to handle the volume produced. But these 71 facilities would only produce 0.03% of the state’s 2015 electricity consumption and 3.2% of its 2015 distillate fuel production and would only sequester 0.2% of its 2015 greenhouse-gas emissions. This study suggests a very limited contribution of biochar to climate mitigation, relative to other options.
Carbon Sequestration Markets
There are only a few private carbon-offset markets available, and none have institutionalized a market for carbon offsets related to biochar. In addition to the study reviewed above, additional studies have estimated what level of carbon-offset income may be generated from biochar production. These are based on estimates of lifecycle greenhouse-gas emissions and price expectations of future unknown carbon prices. One of the distinct advantages of biochar is that it provides a relatively easy measurement for soil-carbon sequestration, compared to other ways of increasing soil-carbon sequestration.
In hopes of making biochar available for private or public carbon-sequestration markets, the International Biochar Initiative (IBI) tried to get biochar recognized by the American Carbon Registry (ACR). However due to insufficient scientific validation of biochar’s ability to remain stable in soils, ACR in 2015 rejected IBI’s application. Furthermore, a separate attempt to get biochar projects in California to provide carbon credits for California’s Cap and Trade Market also failed, despite great effort to develop a biochar-carbon protocol.
Regulatory Issues
The production of biochar has several potential regulatory issues to overcome before a biochar industry can develop. Major issues include the following:
- Applications and potential carbon-dust air pollution. Biochar is very light and easily broken into small particles that can become airborne.
- Air-emission standards from biochar production have not been fully examined and may vary depending on the design of the pyrolysis equipment.
- Water-quality issues related to applied biochar on potentially erodible fields.
- Potential heavy metal content of biochar (depending on the biomass feedstock) and its effect on human and animal health.
While these issues are not beyond solution, they will all have to be investigated and will likely add costs to the production and use of biochar as a soil amendment.
Relationship to Climate Change and Soil Carbon Sequestration
One of the most promising aspects of combined biochar and bioenergy production is that it could be an important renewable-energy source with the potential to significantly mitigate greenhouse-gas emissions and slow climate change. Figure 2 provides an illustration of this capacity of biochar. The percentages in the figure are only estimates of potential atmospheric-carbon offsets. They are not yet fully documented and are used here only as an illustration of the process.
The first illustration shows the carbon-sequestration process. This represents the natural carbon cycle. As plants pull carbon dioxide (CO2) from the atmosphere, part of that carbon is built into the plants’ structures through the process of photosynthesis, while the rest is returned to the atmosphere as CO2 through respiration. When plants shed leaves and branches or die altogether, they add that embodied carbon to the soil. Although most plant above-ground residue carbon is rather quickly released back into the atmosphere as CO2 through soil microbiological activity, root-residue carbon is more efficiently (30 to 35%) converted to longer-term sequestered SOC (Brady and Weil, 2008). Over the long run, relative amounts of CO2 that are sequestered and released in a natural ecosystem are more or less balanced, and hence the process is said to be carbon neutral. Carbon neutral means that there is no net carbon added to the atmosphere, other then what naturally occurs. Climate change is caused in part by net additions of carbon (carbon positive) to the atmosphere. These additions are primarily due to humans burning carbon-based fossil fuel stocks at an increasing rate over the past 500 years. Carbon negative refers to the actual net reduction of carbon in the atmosphere, which can occur in any natural or agricultural ecosystem where gross photosynthesis exceeds the sum of plant and soil (microbial) respiration.
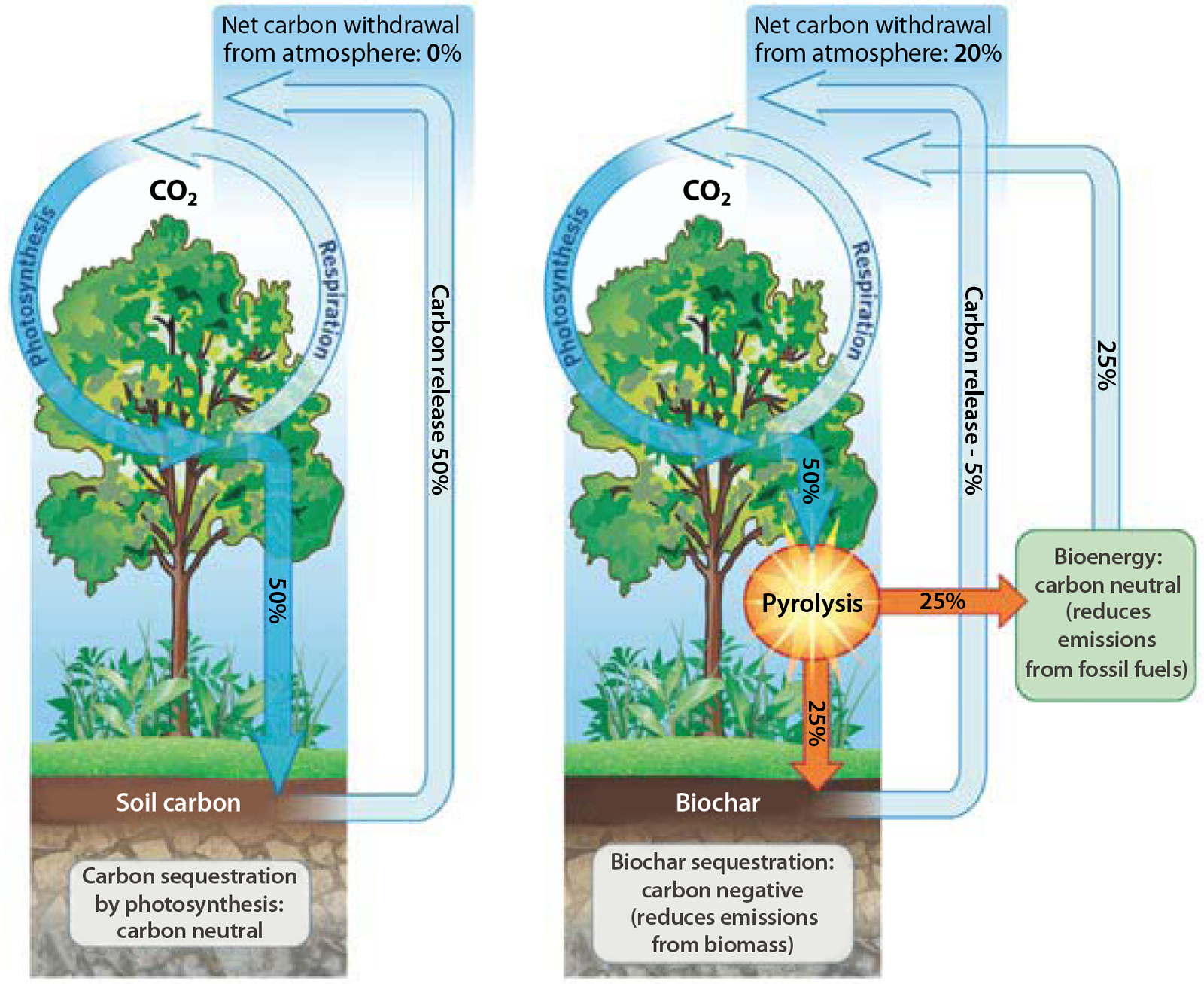
Figure 1. Biomass pyrolysis and best use of its products could be a carbon negative process. Source: International Biochar Initiative
In the case of biochar in Figure 1, the natural process is interrupted by capturing part of the biomass before it reaches the soil directly and using part (25% in the example above) for the production of bioenergy and part for the production of biochar. The illustration shows that the biomass that is converted to energy (in the forms of heat, gas, or liquid fuels) releases part of the carbon in the form of CO2 back into the atmosphere in an assumed carbon-neutral process. The other part of the biomass is converted into biochar and because of its reported stability, may sequester all but 5% of the carbon originally fixed through photosynthesis (in this illustration) in the soil. Thus, use of biochar as a soil amendment can make the whole pyrolysis process a net carbon-negative source of energy.
The ability of combined biochar and bioenergy production to offer carbon-negative renewable fuel through its soil amendment coproduct is limited to critical points in the process of its production and use. First, it is important that biochar applied as a soil amendment remains sequestered for a very long time, and/or contributes to stabilization of SOC from other sources (other organic amendments and plant-biomass residues, especially root biomass and root exudates). In climate-change jargon, this refers to the issue of permanence. In other words, it would be hard to claim a permanent sequestration of carbon if the biochar carbon that was applied as a soil amendment was immediately released back into the atmosphere through possible microbial decomposition processes. Many studies to date indicate that biochar applied to soil releases carbon back into the environment very slowly, with turnover times of several hundreds to thousands of years (Lehmann et al., 2004). However, net C sequestration can vary depending on the impacts of biochar on other fractions of the soil organic carbon (SOC). For example, biochar applications of 13, 26, and 39 tons per acre in a corn-wheat rotation reduced the sequestration of crop residue carbon into SOC over a five year period by ¼, ½, and 2/3, respectively, probably by stimulating residue decomposition (Xinliang et al., 2018). In another study, a one-time biochar application at 10 tons per acre enhanced total SOC and organic N in several cropping systems (continuous corn, corn-soy, corn-soy-triticale, corn-switchgrass, continuous switchgrass), and the benefits increased with time (two to six years) after application (Aller et al., 2017).
Whether or not hundreds or thousands of years means a permanent sequestration, it is a much slower release than the soil carbon sequestration that occurs when agricultural practices such as conservation tillage are adopted as a means to mitigate climate change. For example, continuous no-till can sequester considerable SOC, but it does so in a physically protected form within aggregates near the soil surface; a single tillage pass can release most of this carbon (Cavigelli, 2010; Grandy and Robertson, 2007). It also offers safer and likely less expensive carbon sequestration than methods related to the storage of carbon dioxide in underground geologic formations (known as carbon-capture and sequestration technologies). However, some people argue that other approaches to SOC sequestration, including management-intensive rotational grazing systems, use of finished compost, and restoration of degraded lands to native perennial vegetation may be more effective in achieving a net carbon-negative system than biochar manufacture and use (North, 2015). For example, although a 10-ton-per-acre biochar application led to an increase in topsoil SOC from 2.07 to 2.84% over several years (~7.7 tons per acre C accrual in the plow layer), growing switchgrass without any off-farm inputs raised SOC to 2.56%, representing actual net C sequestration of about 4.6 tons per acre (Aller et al., 2017).
Second, the carbon-negative potential of biochar is either enhanced or limited by the efficiency of energy production and the ability of the overall production process to limit carbon dioxide and other greenhouse-gas emissions. In part, this is because of controversy over the scientific methodologies for measurement of biomass-based energy production (UNEP, 2009). Nonetheless, to understand these potentials for biochar properly, a lifecycle analysis of biochar needs to be examined to account fully for energy efficiency and greenhouse-gas emissions. Lifecycle analysis is a method used to evaluate the environmental burdens associated with a product, process, or activity throughout its full life by quantifying energy, resources, and emissions and assessing their effect on the environment. Only a few researchers have undertaken this type of analysis for biochar. Several have concluded that biochar could result in a net reduction in greenhouse-gas emissions (carbon-negative) and is an energetically efficient use of biomass (Gaunt and Lehmann, 2008; Lehmann and Joseph, 2015; Roberts et al., 2010; Homagain et al., 2016), while at least one has raised major concerns about net ecosystem impacts of biochar manufacture on a large scale (North, 2015).
Biochar may be an efficient source of renewable energy. More specifically, one study estimated that the production of biochar was from two to five times more likely to reduce greenhouse-gas emissions than if the biomass was used just for the production of energy alone (Gaunt and Lehmann, 2008). Significantly, the energy produced per unit of energy input (known as the energy ratio) was estimated to be in the range from 2 to 7, which means that output energy of biochar production is between two and seven times greater than the energy input for its production. This estimated energy ratio for biochar is potentially more energetically efficient than energy production for other biofuels like corn ethanol, or even new technologies such as cellulosic ethanol. Figure 2 provides an example of the details of the lifecycle analysis of biochar, led by Kelli Roberts of Cornell University.
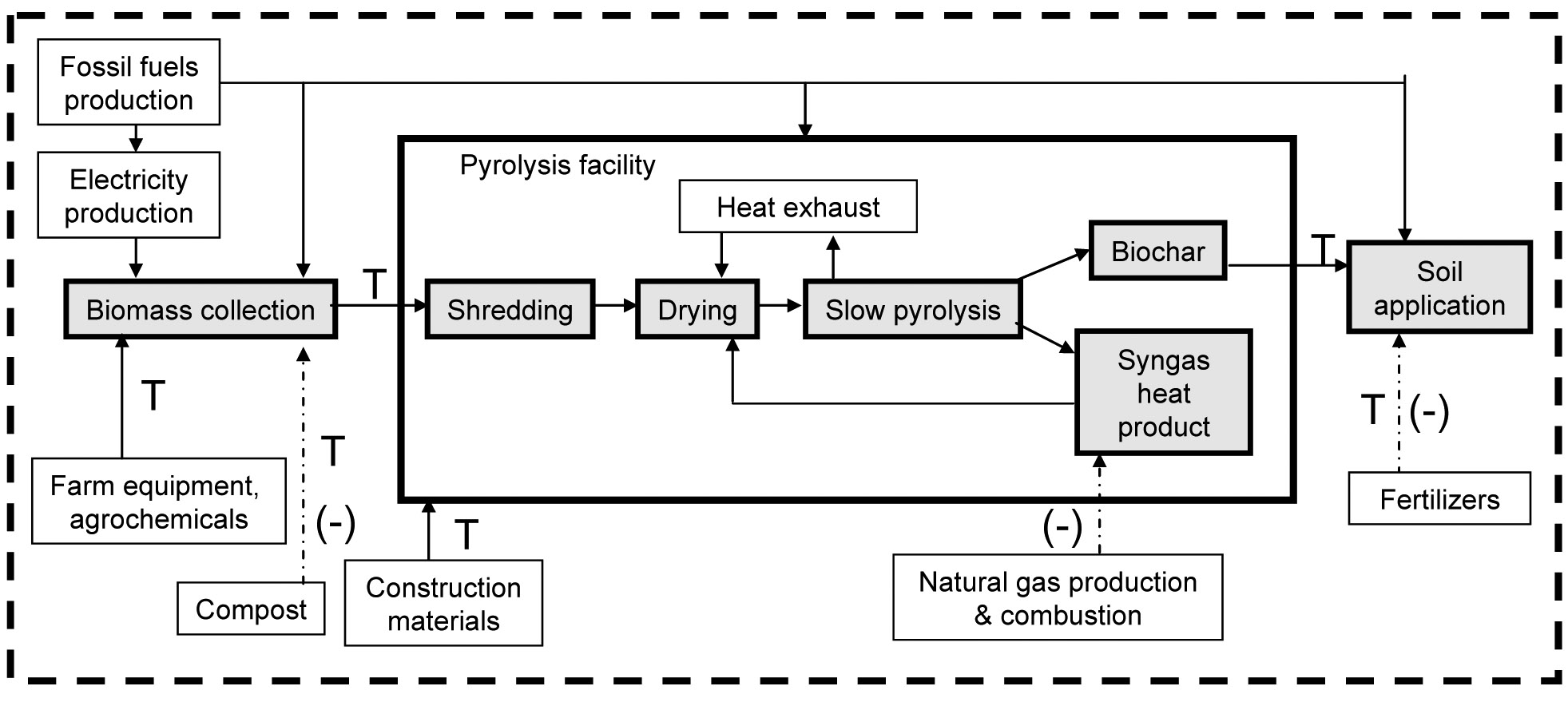
Figure 2. Life-cycle analysis of biochar. Source: Life Cycle Assessment of Biochar Systems: estimating the energetic, economic and climate change potential (Roberts et al., 2010). T=Transportation energy
Finally, these early positive results of lifecycle analysis need further verification and more careful study before anyone can say with great certainty that biochar has the potential to provide carbon-negative renewable energy. This caution is advised for three important reasons.
First, the lifecycle analyses to date are based on research that has yet to clearly demonstrate that biochar applied to all soils can both reduce nitrous oxide (N2O) emissions from soil and enhance fertility. Nitrous oxide emissions from soils represent the single greatest source of greenhouse-gas emissions (in carbon dioxide equivalents or CO2eq) from agriculture production and are related to the application of readily available N from synthetic or organic sources (Charles et al., 2017; Eagle et al., 2017). If biochar can reduce nitrous oxide emissions because it can reduce the need for soluble N fertilizers and/or lower soil nitrous oxide emissions by improving soil N cycling and retention, then biochar production and application to agricultural lands can play that much more of a role in climate change mitigation.
In laboratory incubations, biochar amendments at 1% by volume (~10 tons per acre mixed to plow depth) reduced N2O emissions from a Hanford sandy loam (a bottomland Entisol or young soil of the Central Valley of California) by 74%, roughly equal to the efficacy of a nitrification inhibitor and a urease inhibitor commonly used in conventional N management (Cai et al., 2016). Other research suggests that the majority of the net lifecycle GHG mitigation from biochar use relates to the stable carbon in the biochar, and only a minor part results from reduced N fertilizer use and reduced N2O emissions (Roberts et al., 2010). Additional research is needed to better quantify the net GHG-mitigation benefits of improved N cycling, reduced fertilizer requirements, and reduced soil N2O emissions resulting from biochar amendments.
Second, energetic analyses of cropping systems, which determine how much energy goes into the production of biomass energy crops, are also limited. Thus, it is difficult to know which biomass cropping systems can reduce fossil-fuel use.
Third, because of the high application rates needed for efficacy (typically 10 tons per acre), the sourcing of pyrolysis feedstocks for biochar manufacture raises serious environmental concerns. North (2015) emphasizes that there exist “no biochar feedstocks produced in any ecosystem on the planet whose massive expropriation would not damage the normal necessary function of the carbon cycle in that ecosystem.”
With improved work on these three issues, future lifecycle studies can better measure the carbon-negative fuel capability of the biochar production process.
Limits of Biochar and Climate Change: The Fuel-Versus-Food Debate
Biochar as a potential renewable energy source and a means to mitigate climate change depends on the sustainable use and production of the biomass sources. One major issue that looms for all biomass-based energy—including biochar development—is what is commonly referred to as the fuel-versus-food debate. Environmental and human rights activists have raised serious concerns about “land grabs” in the global south for industrial-scale biochar manufacture enterprises, as well as for direct biofuel production (North, 2015). Another characterization of this debate is what has been called the trilemma of the food, energy, and environment implications of beneficial and sustainable biofuels (Tilman et al., 2009).
This trilemma is related to the general issue of sustainability and how to maximize multiple objectives simultaneously. In the case of beneficial and sustainable bioenergy, the trilemma is posed as follows:
Biofuels done right can be produced in substantial quantities. However, they must be derived from feedstocks produced with much lower lifecycle greenhouse gas emissions than traditional fossil fuels and with little or no competition with food production. (Tilman et al., 2009)
Broadly, how can biofuels be produced in a way that does not over time destroy our natural environment and also does not reduce our ability to maintain and improve food security for all people? The authors who posed this question provided a list of beneficial feedstocks for bioenergy that can address this trilemma (Tilman et al., 2009):
- Perennial plants grown on degraded lands abandoned from agricultural use
- Part of crop residues from agricultural production, provided that a significant portion is returned to land to enhance future soil fertility and health
- Sustainably harvested wood and forest residues
- Double crops and mixed cropping systems that integrate food and dedicated fuel crops
- Municipal and industrial wastes
The authors also point out that the full development of these biomass stocks needs to be done in a way that does not indirectly result in significant land-use changes that can lead to even greater releases of greenhouse-gas emissions. For example, if parts of the crop residues produced in food production are not returned to soils to maintain soil fertility and health, then that loss may be made up with increased synthetic fertilizer use. Extreme caution is needed; for example, removal of above-ground corn residue (stover) can shift the crop rotation from net SOC sequestration to net SOC losses and increased soil erosion (Blanco-Canqui et al., 2016a; 2016b), with a SOC breakeven point at about 30% stover removal (Andrews, 2006). Soil degradation from excessive residue removal can necessitate greater fertilizer inputs, with their embodied energy (CO2 emissions) and increased risk of soil N2O emissions. Furthermore, if food-producing acres are substituted for dedicated energy crops, this may cause the destruction of forests and grasslands in other parts of the world to make up for the lost production of food on those acres. This can lead to an even greater release of greenhouse-gas emissions, as well as aggravating food insecurity in many cases. This last problem is often referred to as the indirect land-use issue and is the source of considerable debate in assessing the sustainability of various biofuel production systems. For more information, see the article Use of U.S. Croplands for Biofuels Increased Greenhouse Gases through Land Use Changes (Searchinger et al., 2008).
There seems to be great attention focused on these issues, as well as international attempts to produce principles of sustainability to use in identifying biomass sources for ultimate biochar conversion. The International Biochar Initiative (IBI) has developed a voluntary biochar certification program based on its biochar standardized production definition and testing guidelines for use in soils. Because biochar efforts are largely directed toward the production of renewable carbon-negative bioenergy (versus simply carbon-neutral fuels), there often is an inherent understanding of these issues among biochar advocates. However IBI’s certification program does not include recognition of the carbon-mitigation impacts of biochar use. Thus, this certification program and label does not provide any information about the sustainability of the harvest of biomass used in its production.
Summary: The Future of Biochar for Sustainable Agriculture
When utilized as one component in an integrated soil-health-building program, biochar has potential for the further development of sustainable agriculture production systems. It could be used as a potential renewable-energy source, as a soil amendment to improve nutrient cycling and fertilizer-use efficiency, and, perhaps most importantly, as a way to mitigate human impacts on the climate. Ongoing research on the many complex issues related to biochar production systems will be needed to more fully understand the implications for food systems, environment protection, and sustainable bioenergy production. Finally, biochar could play an important role in rural economic development because it can be scaled down for smaller communities closer to biomass sources.
It is important to understand that biochar is a tool—one input component within a holistic sustainable or organic system that integrates crop rotation, cover crops, careful tillage, and judicious use of organic inputs including compost as well as biochar itself—to promote soil health and net carbon sequestration. Terra preta, the inspirational ancient-indigenous, agricultural phenomenon that led to the modern biochar industry, is more than black carbon or biochar–it is biochar + humanure + kitchen scraps + ashes + other as-yet-unidentified organic inputs and/or practices that, over the centuries, turned leached-out tropical Oxisols into soils that rival the Corn Belt Mollisols in fertility. Instead of seizing on ONE component of the terra preta phenomenon as a panacea, biochar must be evaluated within the broad context of the integrated system.
Finally, the manufacture and use of biochar must be evaluated for its ecological and social costs (especially sourcing of feedstock), as well as benefits to the recipient acreage. Perhaps the most ecologically sound biochar application might be the careful use of prescribed burning (where appropriate—where the natural ecosystem includes periodic fire) to generate in-situ biochar as part of the soil-building system. The most sociologically sound uses might entail small-scale homestead or community-scale pyrolysis facilities utilized to process locally sourced organic feedstocks that are not otherwise needed to sustain soil or ecosystem health.
Biochar and Sustainable Agriculture
By Jeff Schahczenski
NCAT Agricultural and Natural Resource Economist
Published 2010
Updated January 2018
©NCAT
IP358
This publication is produced by the National Center for Appropriate Technology through the ATTRA Sustainable Agriculture program, under a cooperative agreement with USDA Rural Development.